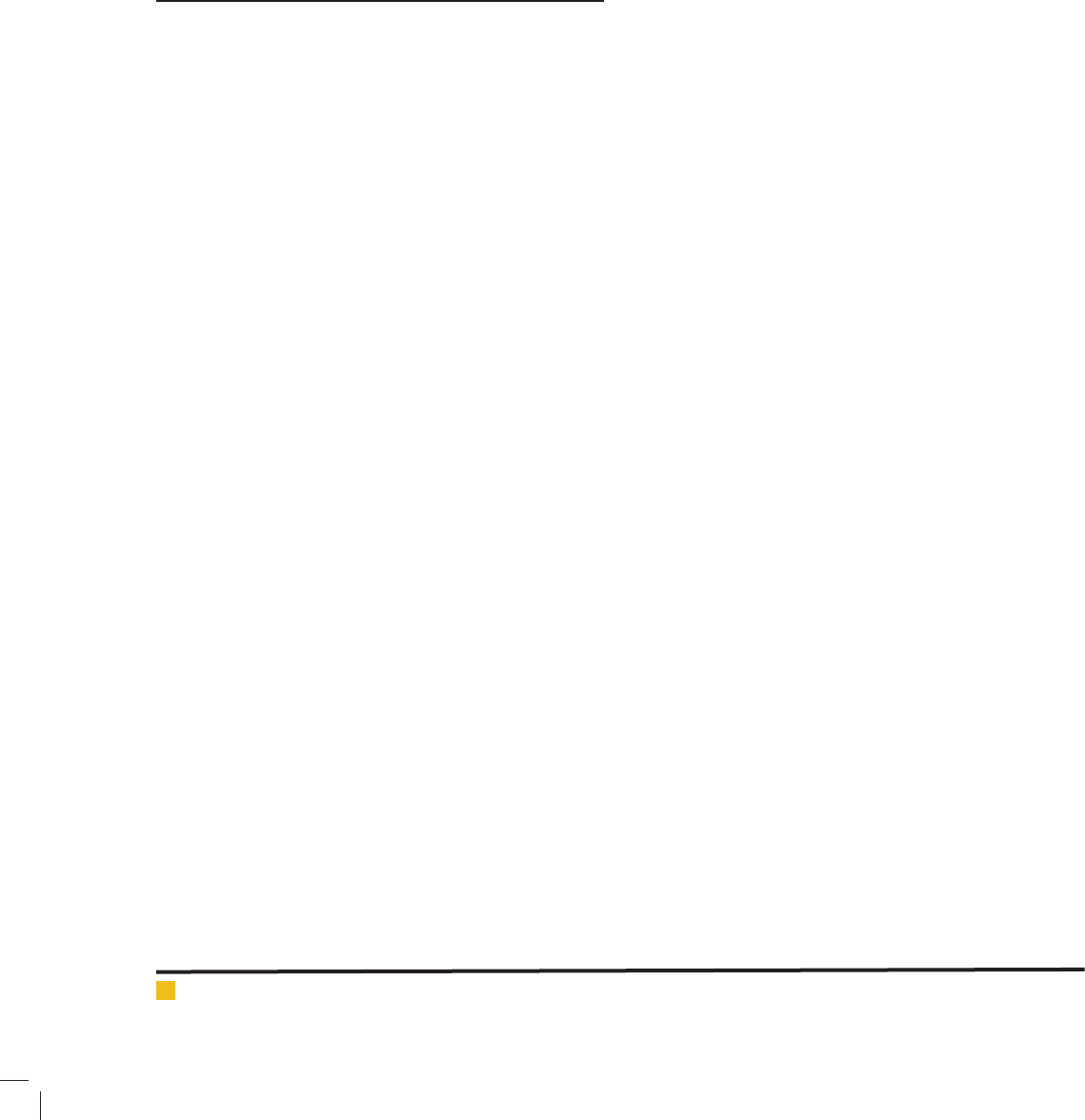
Jie Zhang et al.
364 MUTATION AND PHYTASE THERMAL STABILITY FROM
ESCHERICHIA COLI
BIOSCIENCE BIOTECHNOLOGY RESEARCH COMMUNICATIONS
action might be another reason for the increase of af nity
between phytase and its substrate. The isoelectric point of
Lys and Glu are pH 9.74 and 3.22, respectively. Substitu-
tion of Lys for Glu presumably contributes to the decrease
of the optimum reaction pH. Our results have showed that
phytase AppA mutant had excellent pH stability (Keeping
80% residual enzyme activity from pH 2.0 to 8.0 for 2 h
at 37° C), which is more favorable to apply to animal feed
than wild-type AppA and other phytase (Rocky-Salimi,
et al., 2016).
ACKNOWLEDGMENTS
This work was supported by grants from the Natural
Scienti c Foundation of Shandong Province, China
(ZR2014CM046, ZR2010CQ031 and ZR2015CL019) and
Collaborative Innovation Center of Chinese medicine
antivirus in Shandong University of Tranditional Chi-
nese Medicine (XTCX2014B01-07).
REFERENCES
Cereghino, J. L. and Cregg, J. M. (2000): Heterologous protein
expression in the methylotrophic yeast Pichia pastoris. FEMS
Microbiol Rev, 24, 45-66
Cregg, J. M., Cereghino, J. L., Shi, J. and Higgins, D. R. (2000):
Recombinant protein expression in Pichia pastoris. Mol Bio-
technol, 16, 23-52
Fan, C., Wang, Y., Zheng, C. and Fu, Y. (2013): Fingerprint
motifs of phytases. African Journal of Biotechnology, 12,
1138-1147
Fei, B., Cao, Y., Xu, H., Li, X., Song, T., Fei, Z. and Qiao, D.
(2013): AppA C-terminal plays an important role in its ther-
mostability in Escherichia coli. Curr Microbiol, 66, 374-378
Fei, B., Xu, H., Zhang, F., Li, X., Ma, S., Cao, Y., Xie, J., Qiao,
D. and Cao, Y. (2012): Relationship between Escherichia coli
AppA phytase’s thermostability and salt bridges. J Biosci Bio-
eng, 115, 623-627
Golovan, S., Wang, G., Zhang, J. and Forsberg, C. W. (2000):
Characterization and overproduction of the Escherichia coli
appA encoded bifunctional enzyme that exhibits both phytase
and acid phosphatase activities. Can J Microbiol, 46, 59-71
Greiner, R., Carlsson, N. and Alminger, M. L. (2001): Stere-
ospeci city of myo-inositol hexakisphosphate dephosphoryla-
tion by a phytate-degrading enzyme of Escherichia coli. J Bio-
technol, 84, 53-62
Greiner, R., Konietzny, U. and Jany, K. D. (1993): Puri cation
and characterization of two phytases from Escherichia coli.
Arch Biochem Biophys, 303, 107-113
Guerrero-Olazaran, M., Rodriguez-Blanco, L., Carreon-Trevino,
J. G., Gallegos-Lopez, J. A. and Viader-Salvado, J. M. (2010):
Expression of a Bacillus phytase C gene in Pichia pastoris and
properties of the recombinant enzyme. Appl Environ Micro-
biol, 76, 5601-5608
Haefner, S., Knietsch, A., Scholten, E., Braun, J., Lohscheidt, M.
and Zelder, O. (2005): Biotechnological production and appli-
cations of phytases. Appl Microbiol Biotechnol, 68, 588-597
Kim, M. S. and Lei, X. G. (2008): Enhancing thermostability
of Escherichia coli phytase AppA2 by error-prone PCR. Appl
Microbiol Biotechnol, 79, 69-75
Kim, M. S., Weaver, J. D. and Lei, X. G. (2008): Assembly of
mutations for improving thermostability of Escherichia coli
AppA2 phytase. Appl Microbiol Biotechnol, 79, 751-758
Kim, O. H., Kim, Y. O., Shim, J. H., Jung, Y. S., Jung, W. J.,
Choi, W. C., Lee, H., Lee, S. J., Kim, K. K., Auh, J. H., Kim,
H., Kim, J. W., Oh, T. K. and Oh, B. C. (2010): beta-propeller
phytase hydrolyzes insoluble Ca(2+)-phytate salts and com-
pletely abrogates the ability of phytate to chelate metal ions.
Biochemistry, 49, 10216-10227
Kim, Y. O., Kim, H. W., Lee, J. H., Kim, K. K. and Lee, S. J.
(2006): Molecular cloning of the phytase gene from Citrobacter
braakii and its expression in Saccharomyces cerevisiae. Bio-
technol Lett, 28, 33-38
Leytem, A. B., Widyaratne, G. P. and Thacker, P. A. (2008):
Phosphorus utilization and characterization of ileal digesta
and excreta from broiler chickens fed diets varying in cereal
grain, phosphorus level, and phytase addition. Poult Sci, 87,
2466-2476
Li, Z., Xiong, F., Lin, Q., d’Anjou, M., Daugulis, A. J., Yang, D.
S. and Hew, C. L. (2001): Low-temperature increases the yield
of biologically active herring antifreeze protein in Pichia pas-
toris. Protein Expr Purif, 21, 438-445
Liao, Y., Li, C. M., Chen, H., Wu, Q., Shan, Z. and Han, X. Y.
(2013): Site-directed mutagenesis improves the thermostabil-
ity and catalytic ef ciency of Aspergillus niger N25 phytase
mutated by I44E and T252R. Appl Biochem Biotechnol, 171,
900-915
Liao, Y., Zeng, M., Wu, Z. F., Chen, H., Wang, H. N., Wu, Q.,
Shan, Z. and Han, X. Y. (2012): Improving phytase enzyme
activity in a recombinant phyA mutant phytase from Aspergil-
lus niger N25 by error-prone PCR. Appl Biochem Biotechnol,
166, 549-562
Luo, H., Huang, H., Yang, P., Wang, Y., Yuan, T., Wu, N., Yao,
B. and Fan, Y. (2007): A novel phytase appA from Citrobacter
amalonaticus CGMCC 1696: gene cloning and overexpression
in Pichia pastoris. Curr Microbiol, 55, 185-192
Ming-Hui CHANG, C.-C. Y., Shiuan-Yuh CHIEN, A.B. ARUN
(2008): Expression of recombinant Pichia pastoris X33 phytase
for dephosphorylation of rice bran fermented liquid. Annals of
Microbiology, 58, 6
Noorbatcha, I. A., Sultan, A. M., Salleh, H. M. and Amid, A.
(2013): Understanding thermostability factors of Aspergillus
niger PhyA phytase: a molecular dynamics study. Protein J,
32, 309-316
Pal Roy, M., Mazumdar, D., Dutta, S., Saha, S. P. and Ghosh,
S. (2016): Cloning and Expression of Phytase appA Gene from
Shigella sp. CD2 in Pichia pastoris and Comparison of Proper-
ties with Recombinant Enzyme Expressed in E. coli. PLoS One,
11, e0145745